Nuclear energy fusion combines light atomic nuclei to form a heavier nucleus, releasing vast energy. It’s the process that powers the sun and promises nearly limitless, clean energy for Earth. This article delves into how nuclear fusion works, its advantages, the current state of research, and the challenges ahead.
Table of Contents
Key Takeaways
Nuclear fusion is a cleaner and more sustainable energy source than nuclear fission, primarily producing helium as a byproduct with minimal radioactive waste.
Magnetic confinement fusion and inertial confinement fusion are the two primary methods being researched to achieve controlled fusion reactions, with significant advancements seen in tokamak and stellarator designs.
International collaborations, such as the ITER project, are crucial for advancing fusion energy research and technology, aiming to overcome technical challenges and pave the way for commercial fusion power.
Understanding Nuclear Energy Fusion
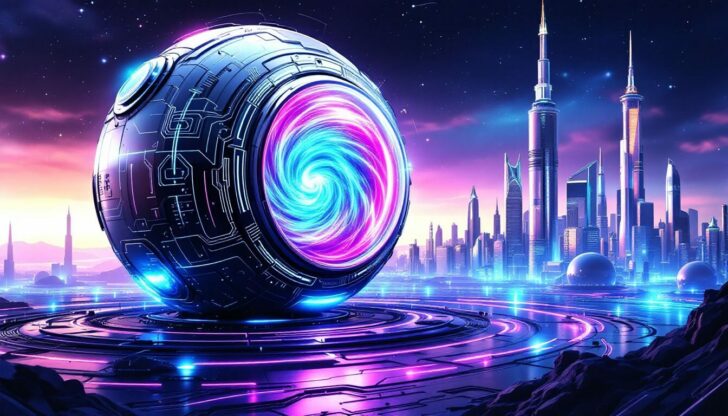
Nuclear fusion occurs when two light atomic nuclei merge to create a heavier nucleus. This process releases a substantial amount of energy. This process is the opposite of nuclear fission, which involves splitting heavy atomic nuclei and has been widely used in power generation for decades. While fusion demands higher initiation temperatures and pressures than fission, it provides a cleaner, more sustainable energy source.
Fusion happens when nuclei overcome repulsive forces to combine, releasing vast energy as heat and light. This energy release happens because the mass of the resultant nucleus is slightly less than the sum of its parts, with the difference being converted into energy according to Einstein’s mass-energy equivalence principle, E=mc². Primary fusion fuels are hydrogen isotopes, deuterium, and tritium, which are abundant and obtainable from seawater and lithium.
Fusion energy offers significant environmental benefits. Unlike nuclear fission, which produces long-lived radioactive waste, fusion primarily produces helium as a byproduct, which is non-toxic and non-radioactive. While tritium, one of the fuels used, is radioactive, it has a relatively short half-life of about 12 years and is used in minimal amounts within fusion reactors. These benefits make fusion a compelling choice for sustainable and environmentally friendly power generation.
The Science Behind Fusion Reactions
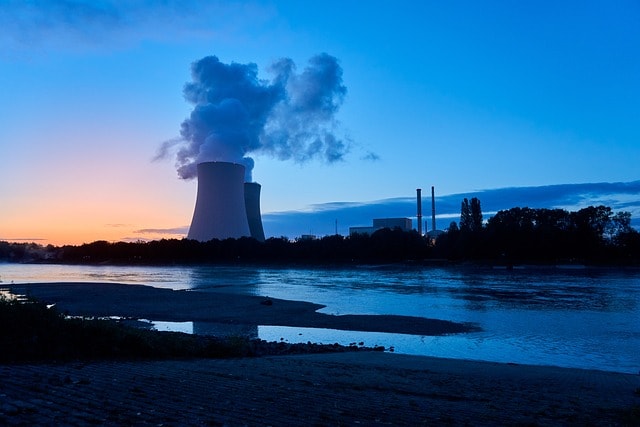
Nuclear fusion necessitates extremely high temperatures and pressures to overcome atomic nuclei repulsion. Typically, fusion reactions necessitate temperatures around 100 million kelvins, which are necessary to provide the kinetic energy needed for the nuclei to collide and fuse. At these extreme temperatures, hydrogen atoms isotopes like deuterium and tritium are transformed into a plasma state, where electrons are stripped from their nuclei.
Fusion reactions involving deuterium and tritium produce a helium nucleus and a high-energy neutron. This reaction releases a significant amount of energy, far greater than that produced by nuclear fission reactions. Fusion energy results from the mass difference between reactants and products, with mass loss converting to energy. This process is known as the D-T fusion reaction and is the most feasible fusion reaction with current technology.
One of the primary challenges in achieving controlled fusion power is maintaining the necessary conditions for a sufficient period. High particle density and confinement time are crucial for increasing the likelihood of collisions between the nuclei, thus sustaining the fusion process.
Researchers have been exploring various methods to achieve and maintain these conditions, including magnetic confinement fusion and inertial confinement fusion, which we will discuss in the following sections.
Magnetic Confinement Fusion Techniques
Magnetic confinement fusion is a technique that uses magnetic fields to confine hot plasma, increasing the likelihood of collisions between atomic nuclei. This method relies on creating and maintaining optimal plasma density, temperature, and confinement time to achieve the conditions necessary for fusion. These systems use toroidal magnetic fields, resembling a doughnut shape, to stabilize and confine plasma.
The Lawson criterion is a fundamental concept in magnetic confinement fusion, establishing the conditions required for a machine to generate sufficient energy. It specifies the optimal combination of plasma density, temperature, and confinement time needed to sustain a fusion reaction. Magnetic confinement systems aim to enhance the confinement properties of plasma, which is essential for achieving practical fusion energy production.
There are two primary designs for magnetic confinement fusion: tokamaks and stellarators. Both designs have their unique advantages and challenges, and significant research is being conducted to improve their performance and feasibility for commercial fusion power plants. Let’s explore these designs in more detail.
Tokamaks
Tokamaks are the most widely researched and developed devices for magnetic confinement fusion. They use a series of magnetic coils arranged around a torus-shaped reactor to create a strong magnetic field that confines the hot plasma within the reactor. The toroidal magnetic field is crucial for maintaining plasma stability and reducing leakage, which increases the confinement time and enhances the chances of fusion reactions occurring.
One of the most ambitious tokamak projects is the International Thermonuclear Experimental Reactor (ITER), which aims to demonstrate the feasibility of fusion energy on a large scale. ITER is designed to produce 500 megawatts of continuous energy output, making it a significant step towards practical fusion power. Recent advancements in high-temperature superconducting devices have also contributed to the development of more compact and efficient tokamaks, improving their potential for commercial use.
In a tokamak, the plasma is heated to about 10 million degrees Celsius, creating the necessary conditions for nuclear fusion. The strength of the magnetic field plays a crucial role in increasing fusion rates, with stronger magnetic fields resulting in higher fusion rates. As research and technology continue to advance, tokamaks hold great promise for the future of fusion energy.
Stellarators
Stellarators are an alternative design for magnetic confinement fusion that offers several advantages over tokamaks. Unlike tokamaks, stellarators do not require a toroidal current to be induced in the plasma, which simplifies their operation and reduces the risk of plasma disruptions. Stellarators are designed to achieve stable plasma confinement using complex magnetic fields generated by external coils.
One of the most notable stellarator projects is the Wendelstein 7-X, which has achieved remarkable results in plasma confinement and stability. The Wendelstein 7-X has reached a maximum plasma temperature of 80 million degrees Celsius, demonstrating its potential for achieving the conditions necessary for fusion. The H-1 stellarator is another important project that aims to explore various plasma configurations and improve magnetic design for fusion power stations.
Stellarators offer a promising alternative to tokamaks, with their unique design potentially overcoming some of the challenges faced by tokamak systems. As research and development continue, stellarators may play a crucial role in the future of fusion energy production.
Learn more, What the return of the stellarator means for fusion energy.
Inertial Confinement Fusion Methods
Inertial confinement fusion (ICF) is another approach to achieving fusion energy, which involves rapidly compressing a small amount of fuel to create the high-pressure conditions necessary for fusion. This method relies on the principle of implosion, where the fuel is compressed to extremely high densities and temperatures, initiating the fusion process. ICF techniques use energetic beams, such as lasers or ion beams, to achieve the required compression and heating.
Magnetized liner inertial fusion (MagLIF) is a technique that combines magnetic fields with inertial confinement to enhance fusion conditions. Incorporating magnetic fields, MagLIF seeks to stabilize and confine plasma during the implosion process. This hybrid approach has shown promising results in achieving the necessary conditions for fusion energy production.
There are two primary methods of inertial confinement fusion: laser-driven fusion and ion beam fusion. Both methods have their unique advantages and challenges, and significant research is being conducted to improve their efficiency and feasibility for practical fusion energy production. Let’s explore these methods in more detail.
Laser-driven Fusion
Laser-driven fusion is primarily achieved through inertial confinement fusion, which utilizes intense laser beams to compress and heat fuel pellets, leading to fusion ignition. The National Ignition Facility (NIF) is one of the leading facilities in this field, using 192 laser beams to compress fuel pellets to extremely high densities and temperatures. In 2022, NIF achieved a significant milestone by successfully demonstrating fusion ignition, marking a major step forward in laser-driven fusion research.
The process involves compressing the core of the fuel to one thousand times liquid density, allowing for fusion reactions to occur effectively. Laser beams provide the necessary energy to compress the fuel pellets, reaching about 30 times solid density. Achieving these conditions requires shorter confinement intervals and higher density, ensuring that the usable triple product values are met. This method has shown great potential for achieving practical fusion energy production.
Globally, there are several notable laser-driven fusion projects, such as the SG-II, HiPER, and Laser Mégajoule. These projects aim to improve laser-driven fusion technologies and contribute to the advancement of fusion energy research. As laser technology continues to evolve, laser-driven fusion holds promise as a viable method for achieving controlled fusion power.
Ion Beam Fusion
Ion beam fusion is another approach within inertial confinement fusion that uses high-energy ions to compress and heat fusion fuel. This method employs heavy ion beams, which are focused by magnetic fields to achieve the required compression and heating conditions for fusion. Heavy ion fusion experiments, such as those conducted with the NDCX-II accelerator, have provided valuable insights into the conditions necessary for practical fusion energy production.
In ion beam fusion, high-energy ions are directed at the fusion fuel, causing rapid compression and heating. This method has shown promise in achieving the extreme conditions needed for fusion reactions, with recent advancements offering new pathways for improving its efficiency and feasibility. The use of high-energy particles in this process ensures that the fuel is heated to the required temperatures and densities for fusion to occur.
The ongoing research and development in ion beam fusion techniques are crucial for advancing our understanding of inertial confinement fusion and its potential for practical fusion energy production. As technology continues to evolve, ion beam fusion may become a key component of future fusion power plants.
Fusion Fuel Sources and Their Availability
The primary fuels for nuclear fusion are deuterium and tritium, which are isotopes of hydrogen. Deuterium is abundantly available and can be extracted from seawater, while tritium is primarily bred in nuclear reactors or obtained from lithium. These fuels are essential for achieving the conditions necessary for fusion reactions and play a crucial role in fusion energy production.
Helium-3 is also considered a potential fusion fuel, offering several advantages over deuterium-tritium fusion. Helium-3 fusion produces fewer radioactive byproducts and has heightened efficiency, making it an attractive option for future fusion energy advancements. The potential for sourcing helium-3 from extraterrestrial bodies, such as the Moon, presents feasible means for its acquisition, enabling future fusion energy advancements.
The availability and benefits of these fusion fuels make them essential for the development and sustainability of fusion power plants. As research and technology continue to advance, the efficient use and sourcing of these fuels will be critical for achieving practical fusion energy production.
Deuterium and Tritium
The fusion of deuterium and tritium is currently the most feasible reaction with existing technology. Deuterium is naturally abundant, occurring in seawater at approximately 30 grams per cubic meter. Tritium, on the other hand, has a half-life of about 12 years, which presents challenges for its handling and storage in fusion reactors. Despite these challenges, tritium can be produced from the reaction of fusion-generated neutrons with lithium, making it a viable fuel for fusion reactors.
When deuterium and tritium nuclei fuse, they result in helium-4 and a free neutron, releasing a significant amount of energy in the process. This reaction, known as the D-T fusion reaction, is considered the easiest and most common in fusion research due to the relatively low temperatures and pressures required compared to other fusion reactions.
The fuel mix used during the JET experiment, which included deuterium and tritium, demonstrated the potential of this reaction for practical fusion energy production. The abundance of deuterium and the ability to breed tritium in a fusion system from lithium underscore the feasibility of using these fuels for fusion power.
As research and development continue, the efficient production and handling of deuterium and tritium will be essential for the success of fusion power plants.
Helium-3
Helium-3 is considered a potential fusion fuel due to its ability to provide clean energy without neutron production. Compared to deuterium-tritium fusion, helium-3 fusion offers several advantages, including reduced radioactive waste and heightened efficiency. The fusion of helium-3 with deuterium produces protons instead of neutrons, which significantly reduces the production of radioactive waste.
The potential for sourcing helium-3 from extraterrestrial bodies, such as the Moon, presents feasible means for its acquisition. Lunar regolith, or moon dust, is known to contain significant amounts of helium-3, making its extraction a viable option for future fusion energy advancements. This extraterrestrial source could provide a sustainable supply of helium-3 for fusion reactors, enabling the development of cleaner and more efficient fusion power plants.
The advantages of helium-3 fusion and its potential availability from lunar sources make it an attractive option for the future of fusion energy. As research and technology continue to advance, helium-3 may play a crucial role in achieving sustainable and environmentally friendly fusion power.
Advances in Fusion Materials and Technologies
Advancements in materials science and technology are critical for developing efficient and effective fusion energy systems. High-temperature superconductors and innovative structural materials play a vital role in improving the performance and durability of fusion reactors. These advancements are essential for achieving the conditions necessary for sustained fusion reactions and ensuring the longevity of fusion power plants.
High-temperature superconductors are being developed to enhance the efficiency of magnetic confinement in fusion reactors, leading to better plasma stability. Innovations in structural materials are also crucial, as they must withstand the extreme conditions inside fusion reactors, such as high temperatures and neutron bombardment. The ongoing research into superconductors and structural materials is essential for advancing the feasibility of commercial fusion energy.
Let’s explore these advancements in more detail, focusing on superconducting magnets and structural materials.
Superconducting Magnets
High-temperature superconducting magnets are being developed to enhance the efficiency of magnetic confinement for fusion reactions. These magnets play a crucial role in confining plasma within fusion reactors, improving plasma stability and increasing the chances of sustained fusion reactions. The SPARC project, for example, utilizes high-temperature superconducting magnets to confine plasma effectively, showcasing the potential of these advanced materials.
One notable development is the YBCO wire produced by SuperOx, which has a conductivity range of 700 to 2000 amps per square millimeter. This high conductivity is essential for creating strong magnetic fields necessary for confining plasma in fusion reactors. SuperOx produced 186 miles of superconducting wire in just nine months, highlighting the rapid advancements in production and the potential for scaling up these technologies.
Advancing high-temperature superconducting magnets marks a major step towards practical fusion energy. As these technologies continue to evolve, they will play a critical role in enhancing the efficiency and feasibility of magnetic confinement fusion reactors.
Structural Materials
Developing structural materials that can endure the intense neutron bombardment and high temperatures within fusion reactors is a significant engineering challenge. Tungsten is considered the optimal material for plasma-facing components in fusion devices due to its high melting point and resistance to neutron damage. However, ongoing research is exploring new materials that can better withstand the severe environments inside fusion reactors.
One of the main issues with using graphite as a plasma-facing material is its high gross erosion rate, tritium redeposition, and potential radiological hazard. As a result, researchers are investigating alternative materials that offer better performance and safety. Innovations in structural materials are vital for the durability and performance of fusion reactors, ensuring that they can operate efficiently under extreme conditions.
The advancements in structural materials are essential for the development of fusion power plants. As research continues, finding materials that can withstand the harsh conditions inside fusion reactors will be crucial for achieving practical and sustainable fusion energy.
Environmental and Safety Considerations
Environmental and safety considerations are critical for the acceptance and success of nuclear fusion as a sustainable energy source. Fusion energy offers significant environmental benefits compared to traditional nuclear fission, producing minimal radioactive waste and having inherent safety features that reduce the risk of large-scale accidents. Effective management of radioactive waste and ensuring the safety of fusion reactors are essential for mitigating any potential environmental impact.
One of the primary environmental benefits of fusion energy is the minimal production of radioactive waste. The main byproduct of fusion reactions is helium, which is non-toxic and non-radioactive. However, the management of tritium, a radioactive isotope used in fusion reactors, is a key concern. Ensuring proper containment and preventing its release into the environment are crucial for minimizing the environmental impact.
Inherent safety features make fusion reactors safer than traditional nuclear fission plants. The risk of a runaway fusion reaction is intrinsically impossible, and there is no risk of a meltdown. If the plasma confinement is disrupted, the fusion reaction ceases almost immediately, preventing any potential hazards. These safety features make fusion reactors an attractive option for future power generation.
Radioactive Waste Management
Effective management of radioactive waste is essential to mitigate the environmental impact of fusion power. The primary byproduct of nuclear fusion is helium, which produces significantly less radioactive waste compared to fission. However, the presence of tritium in fusion reactors poses a potential environmental risk if not properly contained.
Neutron blankets in fusion reactors are designed to absorb high-energy neutrons and heat the blanket, reducing the potential for radioactive waste. With long-term use, each atom in the wall materials of fusion reactors is bombarded by neutrons. As a result, they are displaced approximately 100 times before being replaced. High-energy neutron collisions with wall atoms produce unstable isotopes, which decay by emitting alpha particles, protons, or gamma rays.
The ongoing research and development in radioactive waste management are crucial for minimizing the environmental impact of fusion energy. Ensuring proper containment and disposal of radioactive materials will be essential for the acceptance and sustainability of fusion power plants.
Safety Features of Fusion Reactors
Fusion reactors are built to be inherently safe, with several features that reduce the risk of large-scale accidents. Unlike traditional nuclear fission reactors, fusion reactors cannot experience a runaway reaction, making them intrinsically safer. The energy production process in fusion reactors is designed to prevent any potential hazards.
One of the key safety features of fusion reactors is the immediate cessation of the fusion reaction if plasma confinement is disrupted. This means that in the event of any issues, the fusion reaction stops almost instantaneously, preventing any risk of a meltdown. The lack of a sustained chain reaction in fusion reactors further enhances their safety profile.
In future fusion reactors, there is no risk of meltdown, making them a safer alternative to traditional nuclear fission plants. These inherent safety features contribute to the attractiveness of fusion energy as a reliable and secure source of power for the future.
Major Fusion Research Projects and Collaborations
Fusion research is conducted globally, with significant programs in Europe, Japan, China, India, Russia, South Korea, and the USA. These international collaborations are essential for advancing fusion science and technology, sharing knowledge, and pooling resources to overcome the challenges of achieving practical fusion energy. Major research projects and collaborations play a crucial role in driving the field forward and demonstrating the feasibility of fusion energy.
Toroidal confinement systems have several key components. These include tokamaks, stellarators, and reversed field pinch (RFP) devices. These designs are being explored and developed to improve plasma confinement and achieve the conditions necessary for sustained fusion reactions. Significant projects, such as the H-1 stellarator and the Wendelstein 7-X, have made notable contributions to fusion research, advancing our understanding of plasma behavior and magnetic confinement.
Let’s explore some of the major fusion research projects and collaborations, starting with the International Thermonuclear Experimental Reactor (ITER).
ITER
The International Thermonuclear Experimental Reactor (ITER) is one of the most ambitious and significant fusion research projects in the world. Launched in 1985, ITER is a collaborative international project aiming to demonstrate the feasibility of fusion energy. The project is located in Cadarache, southern France, with construction having begun in 2007.
ITER’s goal is to produce about 500 megawatts of continuous energy output, making it a significant step towards practical fusion power. The project brings together multiple countries, including the European Union, the USA, Russia, Japan, China, South Korea, and India, to collaborate on this landmark effort. India’s inclusion as the seventh member of the ITER consortium highlights the global commitment to advancing fusion energy.
The expected timeline for ITER aims for operational plasma by the 2020s, with the project playing a crucial role in demonstrating the potential of fusion energy as a viable and sustainable power source. ITER represents a major milestone in fusion research and international collaboration.
Other Notable Projects
In addition to ITER, several other notable fusion research projects are making significant contributions to the advancement of fusion technology. The Joint European Torus (JET), located in the UK, is the world’s largest tokamak and Europe’s flagship experiment. JET aims to study fusion power and prepare for ITER by providing valuable data and insights into plasma behavior and confinement.
The Z machine, operated by Sandia National Laboratories in the USA, achieves fusion conditions by creating a powerful electrical pulse that compresses plasma through a Z-pinch effect. This process produces intense X-ray radiation, which is used to study the fusion process and improve our understanding of plasma dynamics.
General Fusion, a private company, aims to develop a large-scale demonstration fusion power plant relevant for power generation by 2025, showcasing the potential for commercial fusion energy and fusion powers.
These projects, along with many others around the world, highlight the global efforts to advance fusion research and technology. Each project contributes to our understanding of fusion energy and brings us closer to achieving practical and sustainable fusion power.
Challenges and Future Prospects
Nuclear fusion faces significant technical and engineering challenges that must be addressed to harness its full potential. Despite these challenges, the prospects for fusion energy remain promising, with ongoing research and development driving advancements in science and technology. The International Atomic Energy Agency (IAEA) plays a crucial role as a supporter of international fusion research and development, facilitating collaboration and knowledge sharing among countries.
One of the primary challenges in achieving successful fusion reactor designs is heating the fuel to around 100 million kelvins, which is necessary to initiate and sustain fusion reactions. Additionally, handling tritium poses challenges due to its short half-life and the difficulties in finding, producing, and storing it. To overcome these challenges, researchers are exploring various methods, such as superconducting magnets, to reduce the high auxiliary power requirements of today’s tokamaks.
The future prospects for fusion energy are promising, with plans in place to develop pilot fusion plants and commercial reactors. The roadmap to commercialization includes timelines for power generation and the potential for fusion to replace conventional energy sources like coal. Let’s delve into these challenges and future prospects in more detail.
Technical and Engineering Hurdles
Fusion energy is an attractive alternative to fossil fuels because it is carbon-free and could potentially yield four times more energy per kilogram of fuel compared to fission. However, a major challenge in achieving successful fusion reactor designs is heating the fuel to around 100 million kelvins, which is necessary to initiate and sustain fusion reactions. This extreme temperature is required to provide the kinetic energy needed for the nuclei to overcome their repulsive forces and fuse.
Handling tritium poses significant challenges due to its short half-life and the difficulties associated with finding, producing, and storing it. Tritium is essential for the D-T fusion reaction, but its radioactive nature and limited availability necessitate careful management and innovative production methods.
Research into superconducting magnets is underway to reduce the high auxiliary power requirements of today’s tokamaks, which is another critical hurdle that must be addressed to make fusion energy commercially viable.
Diagnostic systems in commercial fusion reactors face challenges due to harsher operating environments compared to scientific reactors. Ensuring accurate and reliable diagnostics is essential for monitoring and controlling the fusion process. Despite these challenges, the primary aim of the ITER project is to prove that fusion could produce useful energy, paving the way for future electricity-producing demonstration fusion power plants referred to as DEMOs.
Roadmap to Commercialization
The roadmap to commercializing fusion energy involves several key milestones and timelines:
The European fusion program aims for power generation around the middle of the 21st century.
Plans to develop pilot fusion plants and commercial reactors are underway.
A midsize tokamak prototype named SPARC is expected to be completed in 2025, representing a significant step towards achieving practical fusion energy.
Following the completion of pilot fusion plants, it is estimated that commercial reactors will take about a decade to develop.
Plans are in place to develop fusion power facilities on existing conventional energy sites to streamline the transition to fusion energy. The likely first source fusion plants will replace is coal plants, providing a cleaner and more sustainable alternative for power generation. Companies like Tokamak Energy aim to commercialize fusion by 2030, highlighting the potential for rapid advancements in the field.
The transition to commercial fusion energy requires continued investment in research and development, as well as international collaboration to overcome the technical and engineering challenges. As these milestones are achieved, fusion energy holds the promise of transforming the global energy landscape, providing a clean, safe, and virtually limitless source of power for the future.
Summary
In summary, nuclear fusion represents a promising future energy source with the potential to provide clean, safe, and virtually limitless power. The fundamental science behind fusion reactions, including the fusion of deuterium and tritium, offers significant advantages over traditional nuclear fission, including minimal radioactive waste and inherent safety features. Advancements in magnetic confinement fusion techniques, such as tokamaks and stellarators, and inertial confinement fusion methods, like laser-driven and ion beam fusion, are driving the field forward.
The development of fusion fuel sources, particularly deuterium, tritium, and helium-3, is crucial for achieving practical fusion energy production. Innovations in fusion materials and technologies, including high-temperature superconducting magnets and advanced structural materials, play a vital role in improving the efficiency and durability of fusion reactors. Environmental and safety considerations further emphasize the benefits of fusion energy, making it an attractive alternative to current power generation methods.
Major fusion research projects and international collaborations, such as ITER and JET, are making significant strides in demonstrating the feasibility of fusion energy. Despite the technical and engineering challenges, the roadmap to commercialization presents a clear path towards achieving practical fusion power. Continued investment and international cooperation are essential for realizing the full potential of fusion energy and transforming the future of global energy production.
Frequently Asked Questions
What is nuclear fusion, and how does it differ from nuclear fission?
Nuclear fusion involves the merging of light atomic nuclei to create a heavier nucleus, releasing energy in the process, whereas nuclear fission is the division of heavy atomic nuclei into lighter ones, also resulting in energy release. Thus, fusion is characterized by combining elements, while fission is defined by their separation.
What are the primary fuels used in fusion reactions?
The primary fuels used in fusion reactions are deuterium, sourced from seawater, and tritium, which is bred from lithium or obtained from nuclear reactors. These fuels are essential for achieving the conditions necessary for fusion to occur.
What are the main challenges in achieving practical fusion energy?
Achieving practical fusion energy faces significant challenges such as heating the fuel to extreme temperatures, maintaining plasma stability, and managing tritium effectively. These obstacles must be addressed to advance fusion technology.
What are the environmental benefits of fusion energy?
Fusion energy offers significant environmental benefits, including the production of minimal radioactive waste and enhanced safety features compared to traditional nuclear fission. This positions fusion as a cleaner and more sustainable energy option.
What is the ITER project, and why is it significant?
The ITER project is a significant international collaboration focused on demonstrating the viability of fusion energy by aiming to produce 500 megawatts of continuous energy output. Its success could revolutionize energy production, providing a sustainable and powerful energy source for the future.